Temperature
Feeling the Heat
The heat receptor TRPV1 and the cold receptor TRPM8 aren’t the only molecules of their ilk. Since Julius and Patapoutian’s Nobel-winning discoveries, scientists have identified close to a dozen mammalian receptors that act like tiny thermometers. Some of them are found in sensory neurons and play a role in how we perceive temperatures, from glacially cold to fiery hot. Each receptor – and the neurons that express it – is tuned to a different temperature range, allowing us to tell a balmy breeze from an icy gale or a tepid sip of tea from a scalding gulp.
Natural Mimics
Ever wonder why eating chilies makes you sweat or chopping onions makes you cry? Capsaicin, the pungent chemical in chili peppers, and syn-propanethial S-oxide, which onions release as they are sliced, activate the same sensory receptors in your tongue or eyes that respond to painful heat, causing a sensation of burning. “Everyone thinks we taste spiciness, but we don’t,” Julius says. “We feel it.” Many other substances produced by plants or animals – including the menthol in mint and certain toxins in the venom of spiders and scorpions – similarly trigger feelings of fieriness, warmth, or coolness, depending on which temperature sensors they stimulate.
Touch
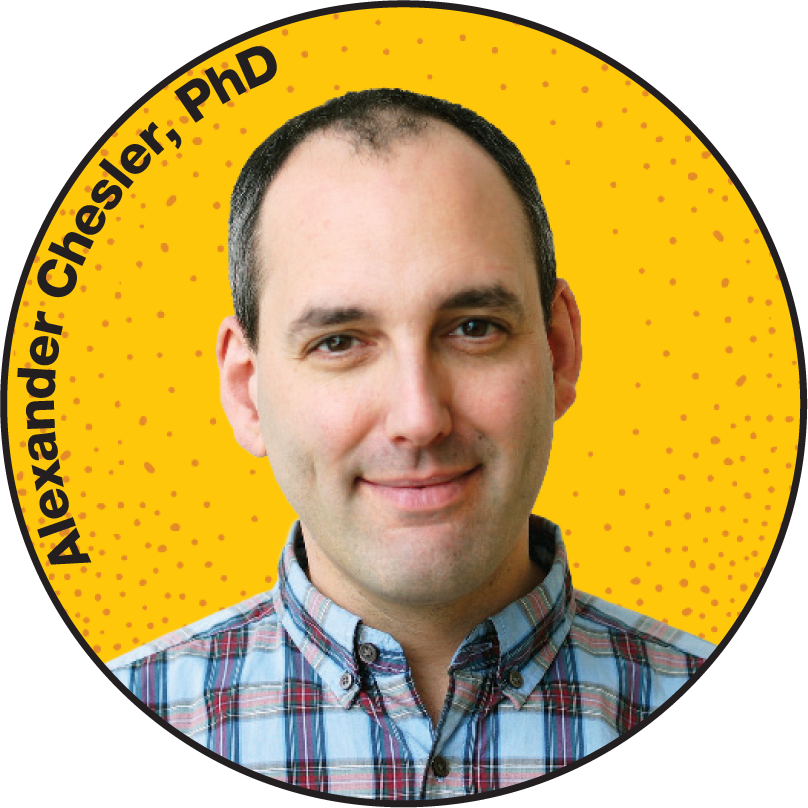
Under Pressure
Arguably our most intimate sense, physical touch lets us feel the embrace of a lover, the brush of a cat’s tail, or a tap on our shoulder. We experience such mechanical encounters thanks to piezo channels, sensory receptors that are unlike any others in our bodies. “They are quite extraordinary,” says Julius lab alum Alexander Chesler of the NIH. “If you take your fingers and rub them together, think about how light that force is. How can we possibly detect that? It’s because of the biophysical properties of these very beautiful and unusual molecules.”
How Piezos Work
A piezo molecule forms a pore, or channel, encircled by propeller-like blades in the membrane of a sensory nerve fiber. “The current thinking is that the blades grab the membrane and curve it around the channel,” like a wound spring, Chesler explains.
The spring’s tension keeps the channel closed until a poke or pull stretches the membrane and thereby unwinds the spring. As a result, the channel opens, initiating a signal to the brain. (See “The Role of Receptors.”)
Illustrations: Chris Philpot
Internal Impressions
Piezo channels don’t just detect external forces. They also give rise to internal sensations, including proprioception (your awareness of where your limbs are in space) and interoception (your awareness of bodily functions, such as your heartbeat, breathing, or digestion).
In collaboration with Patapoutian, Chesler now studies patients who have inherited defects in the gene for PIEZO2, one of two known piezo channels in humans. These rare mutations cause a surprising assortment of symptoms. Patients can’t feel gentle touches or vibrations, nor can they feel their lungs inflating or their bladders filling. “They have to schedule going to the bathroom because by the time they feel it, it’s usually too late,” Patapoutian says. Strangely, though, such individuals can still detect painful forces. “If you pinch them, they can feel it,” Chesler says. (See “The Mystery of Mechanical Pain.”)
People with PIEZO2 mutations also are highly uncoordinated, which makes it difficult for them to walk, and they may suffer muscle or skeletal problems, such as scoliosis, hip dysplasia, or club feet. Most of them were born breech. “Even the coordination of turning around in the birth canal requires proprioception,” Chesler says.
Such insights are challenging assumptions about the role of piezo channels and other sensory receptors in development and disease. “Most clinicians look at these patients and say, ‘This is a motor neuron disease,’” Patapoutian says. “It’s not – it’s a sensory disease. Almost everywhere we look, we are finding that mechanosensing is playing some important function we take for granted.”
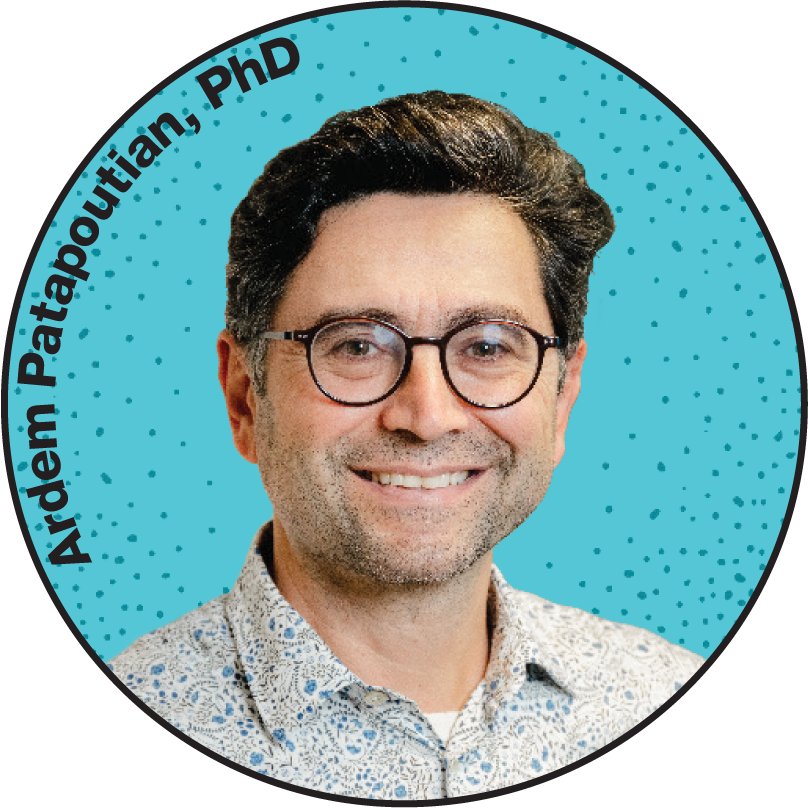
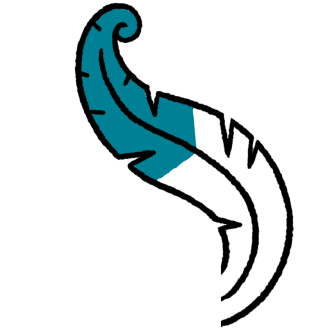
How Nerves Work
Sensory Smorgasbord
Sensory neurons come in many “flavors.” Different types respond to different stimuli – kisses, cuts, tickles, burns, baths, bug bites, buzzing cell phones, and on and on – and their specialties can be remarkably nuanced. “There are touch neurons, for example, that sense a hair moving and others that sense a hair being pulled,” explains Chesler of the NIH. Some neurons sense only one stimulus, such as an itch or a pinch, while others sense multiple stimuli, such as both the coolness and the caress of a fall breeze. One class of sensory neurons, known as polymodal nociceptors, responds to just about anything that hurts. “You can burn your hand, or somebody slaps you – doesn’t matter,” Chesler says. “They’ll respond no matter what it is, as long as it’s nasty.”
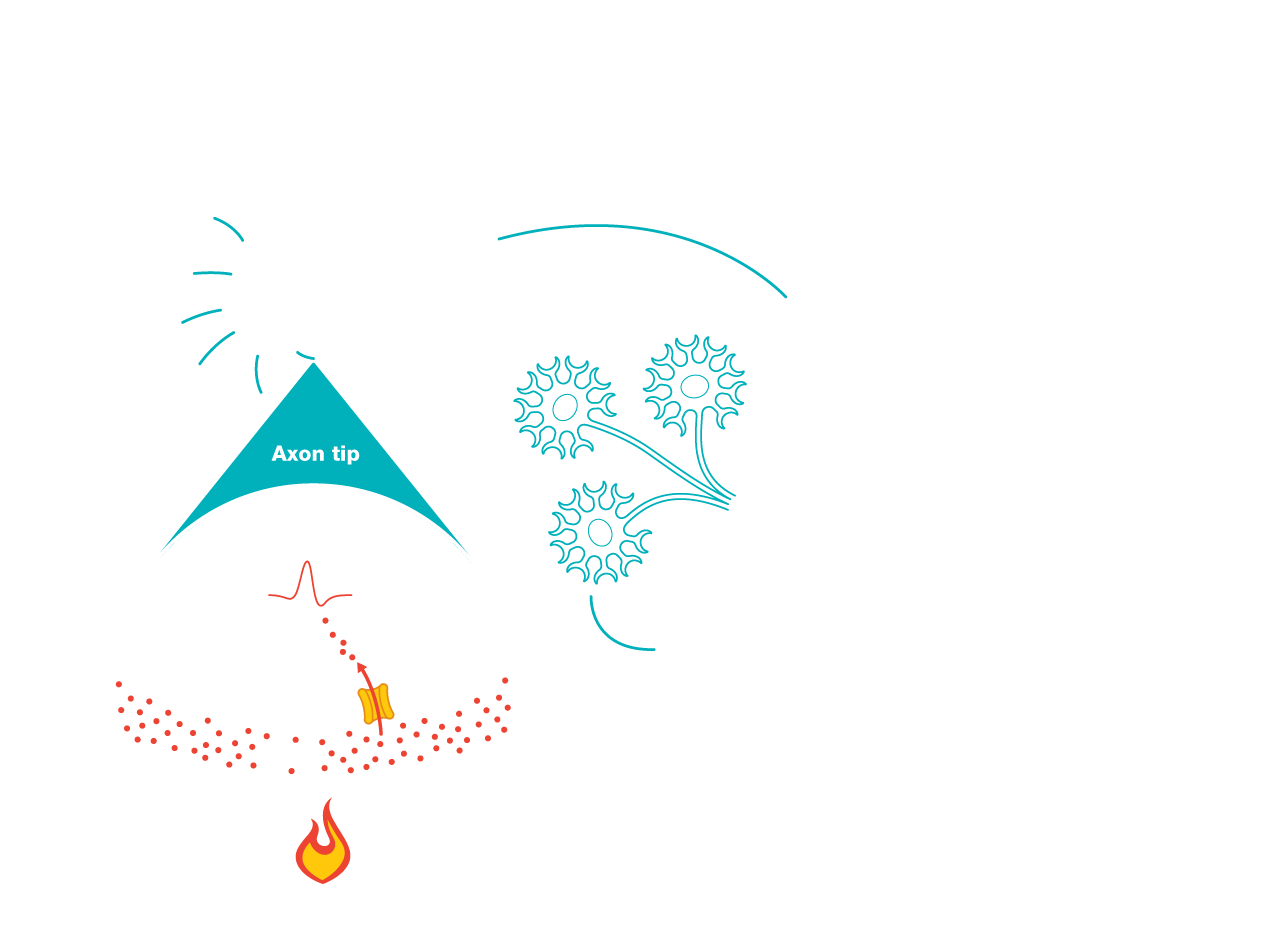
The Role of Receptors
Receptors enable sensory neurons to detect physical stimuli by converting them into electrical signals. These receptor molecules act as gates in the tips of axons, or nerve fibers. When a receptor is activated – such as by heat, pressure, or a chemical reaction – its gate opens. This allows charged particles called ions to flow into a neuron, creating an electrical current that sets off a signaling cascade.
Illustration: Chris Philpot
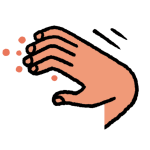
Itch
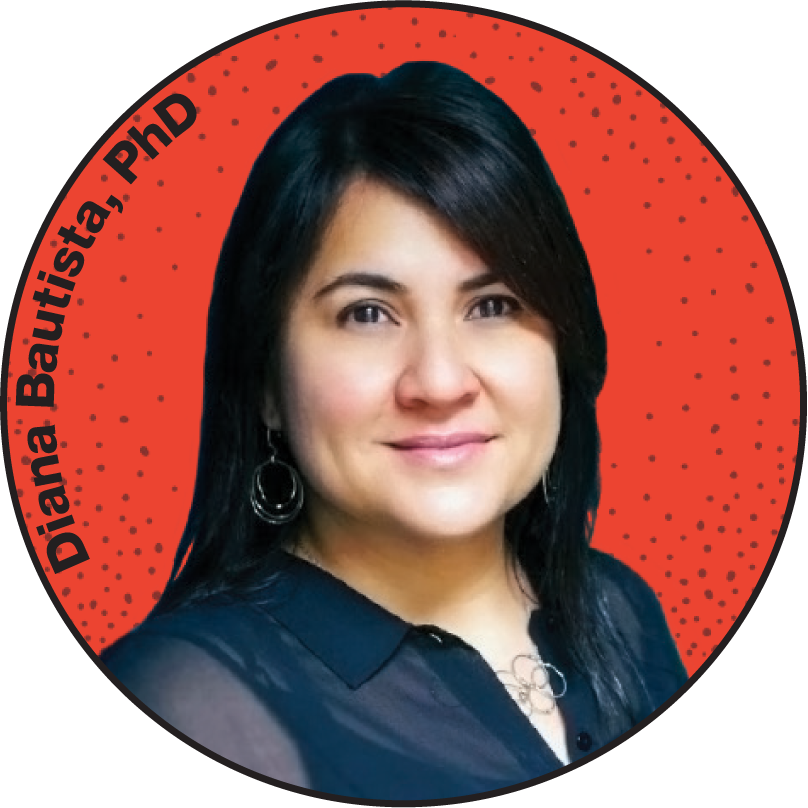
Why We Scratch
“It was thought for a long time that itch was just a mild form of pain,” says Diana Bautista, PhD. More than 15 years ago, as a fellow in Julius’s lab, she showed that the sensory receptor TRPA1 responds to wasabi and other substances that evoke pain. Now a neuroscientist at UC Berkeley, she studies the molecular underpinnings of itch, which are, in fact, very different from those of pain. Itchy things excite different sensory neurons than painful things, Bautista points out, and interact with sensory receptors in different ways.
Her lab has found, for example, that in mouse models of eczema, itchy chemicals called pruritogens can activate TRPA1 and other sensory receptors directly, the way pain stimuli do, as well as indirectly, through a “complex wave of activity” involving many cells and signaling pathways. “Eczema is the most common chronic itch disorder,” Bautista says, and current treatments like antihistamines and corticosteroids are often ineffective. “There is a real need for new therapeutics; we think these receptors could be potential targets.”
Pain
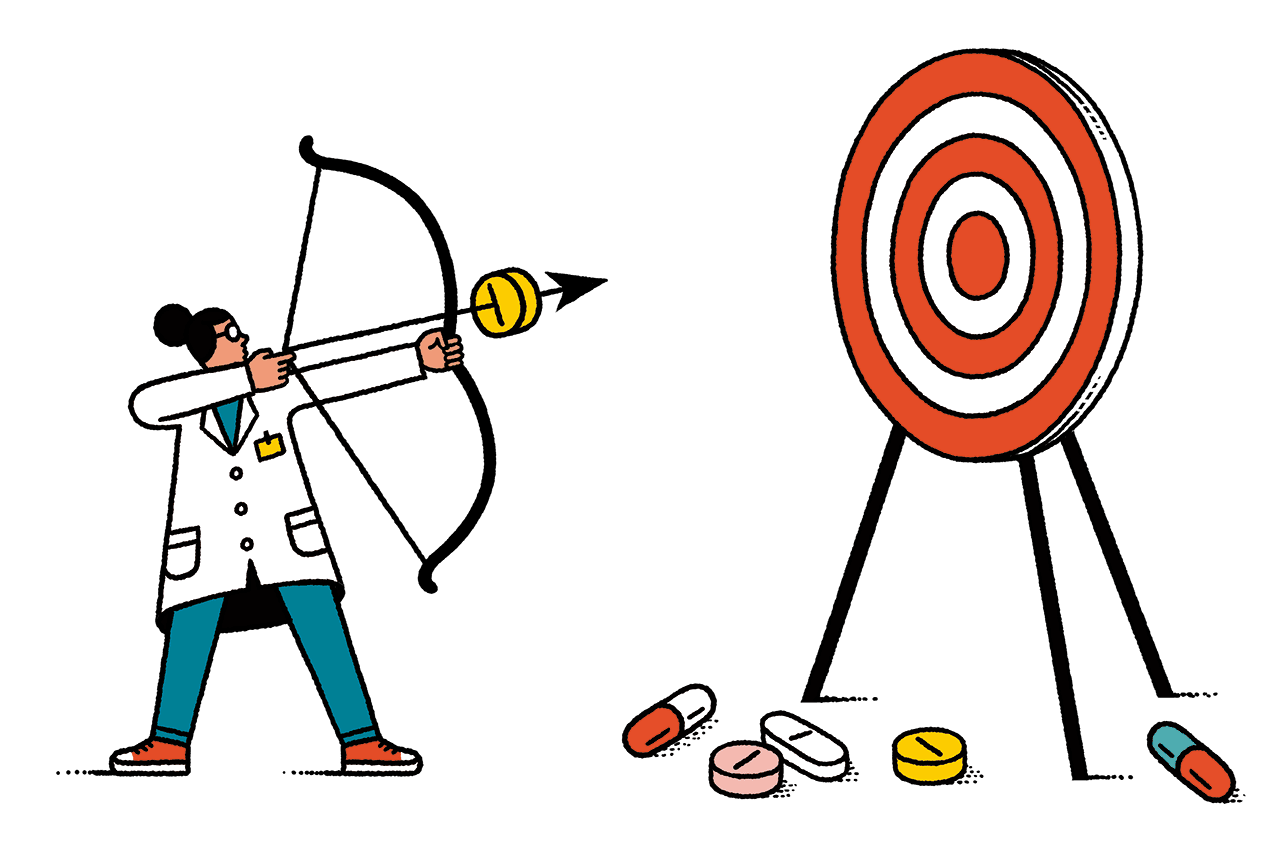
The Promise of Better Painkillers
The holy grail of pain medicine is a drug that can alleviate agony without creating trouble elsewhere in the body. The problem with opioids, for instance, is that they act indiscriminately. Opioids not only numb nerves but also activate the brain’s reward centers (which causes a sense of euphoria that can lead to addiction) and suppress respiration (which is why an overdose can be fatal). “The pill doesn’t know where to go,” explains Allan Basbaum, PhD, a UCSF neuroscientist who specializes in pain physiology.
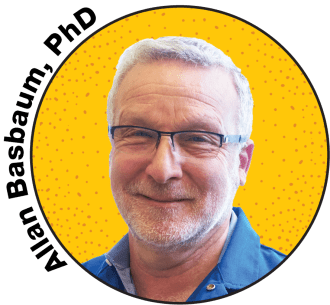
So when Julius’s lab discovered the capsaicin receptor, TRPV1, Basbaum says, the pain field was ecstatic. TRPV1 does more than trigger sensations of heat. It also plays a role in pain caused by inflammation, a hallmark of chronic conditions like arthritis, asthma, and inflammatory bowel disease. Most importantly, the receptor is found primarily in peripheral sensory neurons – those outside the central nervous system. Many pain scientists therefore believed that TRPV1 would lead them to an analgesic drug with few, if any, dangerous side effects.
Finding that drug, however, has been harder than they had thought. “Many groups tried to go after it and failed,” Basbaum says. Some candidates that made it to clinical trials flopped at relieving pain as well as animal models had predicted; others caused slight fevers or increased the risk of burn injuries because they prevented patients from feeling anything hot. But experts haven’t lost hope. Several promising compounds are still in various stages of testing, Basbaum says, and recent advances in molecular imaging may enable scientists to find ways of targeting TRPV1 that allay pain without interfering with important functions, like regulating body temperature and sensing heat. (See “How the Chili Pepper Sparked a Breakthrough in Molecular Imaging.”)
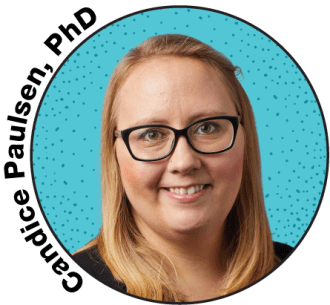
Other receptors in peripheral neurons besides TRPV1 could also make good targets for new pain drugs. Blocking the cold receptor TRPM8, for example, could bring relief to cancer patients taking the drug oxaliplatin, which causes hypersensitivity to cold. Another receptor, TRPA1, is especially intriguing. It responds to all sorts of piquant and painful things – garlic, wasabi, wildfire smoke, animal venoms, tear gas, and the byproducts of chemotherapy drugs, among other substances – and has been implicated in a variety of pain syndromes, including diabetic neuropathy, sickle cell disease, and a rare genetic disorder called familial episodic pain syndrome. Biochemist Candice Paulsen, PhD, an alum of Julius’s lab who now studies TRPA1 in her lab at Yale, calls it “a gatekeeper to the development of chronic pain.” Like TRPV1, the receptor reacts to inflammation but, unlike TRPV1, is not involved in regulating body heat. “The hope is that TRPA1 may be a better drug target because it doesn’t have the temperature side effects,” Paulsen says.
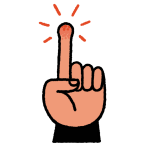
The Mystery of Mechanical Pain
The most frequent form of pain is mechanical – stubbing your toe, say, or slicing your finger, or slipping a disc. Some injuries, including nerve damage caused by conditions like multiple sclerosis and stroke, can cause what’s known as hyperalgesia, in which pain becomes more intense. In many cases of chronic mechanical pain, such as back or knee pain, even slight movements hurt considerably. Scientists have yet to find a mechanism that explains these phenomena. “It’s still a big question mark,” Patapoutian says.
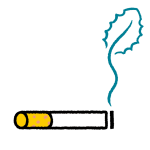
The Painful Truth About Menthol Cigarettes
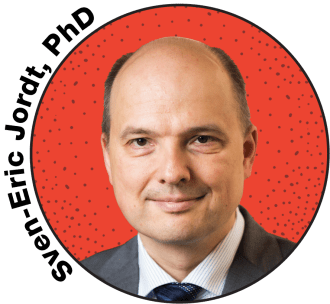
Menthol cigarettes are the most popular kind of smokes – and not just because menthol is a nice flavor, says Sven-Eric Jordt, PhD, a biochemist and physiologist at Duke University and an alum of Julius’s lab. “It’s a potent pharmacological agent,” he explains; the menthol activates cold-sensing receptors in sensory neurons, thereby suppressing pain and cough. Jordt’s research shows that menthol causes mice exposed to cigarette smoke to breathe quicker and thus absorb more nicotine into their bloodstreams. “Menthol makes cigarettes more palatable,” he says. “You inhale more nicotine, and you get addicted faster.”
And now, makers of e-cigarettes are sneaking menthol-like chemicals into their products, Jordt says. “They’re adding these cooling chemicals to candy and fruit flavors that kids like.” While the synthetic mimics don’t share menthol’s minty taste or smell, he points out, they have a similar addictive effect.
Hidden Worlds of Hurt
Surprisingly, neurons are not the only cells in your body involved in pain. Do your teeth twinge when you bite into ice cream? A recent study suggests that tooth cells called odontoblasts could be the culprit. These cells express the cold receptor TRPC5, the activation of which may make teeth extra sensitive to frosty food or drink, particularly when they are inflamed.
Enterochromaffin cells, cells in the lining of the digestive tract, are another suspected pain accomplice. Known as EC cells, they express the receptor TRPA1, which responds to mustard, radishes, and other spicy fare, explains James Bayrer, MD, PhD, a pediatric gastroenterologist at UCSF. Working with Julius’s lab and the lab of Holly Ingraham, PhD, UCSF’s Herzstein Professor of Molecular Physiology (and Julius’s wife), he has found that EC cells communicate with neurons to amplify sensory signals from the gut. When they’re activated, they can make normally benign sensations – such as a stretch of your intestine as food passes through it – feel painful.
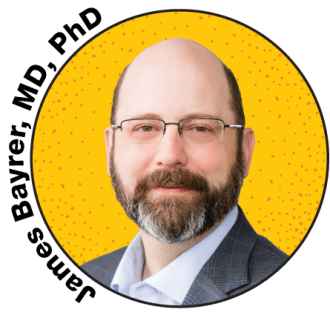
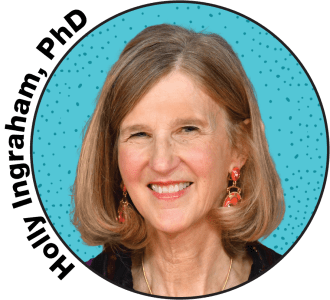
Bayrer suspects this signal amplification is behind abdominal pain disorders like irritable bowel syndrome, which afflicts up to 30% of people and lacks a silver-bullet treatment. “If we could use what we’re learning to find a drug that could turn the volume knob down on the pain,” he says, “that would be fantastic.”
How It Becomes Chronic
Many pathways play a role in chronic pain. Here is one way that sensory receptors, including TRPV1 and TRPA1, can contribute.
This process, called sensitization, can make harmless stimuli, like warmth or gentle touches, feel painful. Some forms of chronic pain develop when sensitization occurs in the absence of an injury – or continues after an injury has healed.
Resolution Revolution
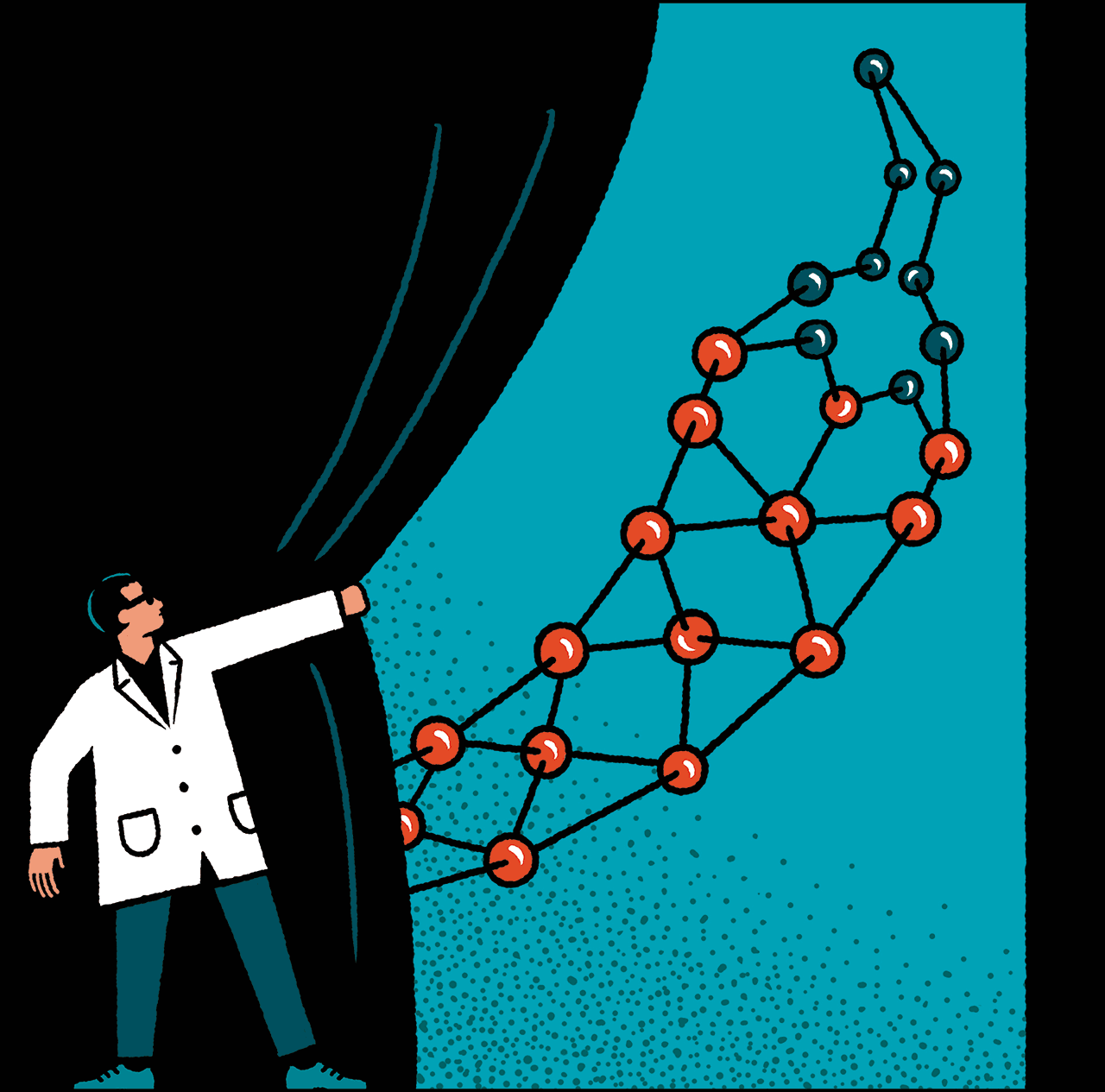
How The Chili Pepper Sparked A Breakthrough In Molecular Imaging
About a decade after Julius made history by locating the capsaicin receptor, the molecule in human nerve cells that gives chilies their kick, his team had examined it in every way they could think of – except for one. They had cloned the gene that codes for it. They had bombarded it with all matter of stimuli to see what sets it off. They had even mutated it in mice and created animals that were immune to spice and some amount of heat and pain. “They had studied this protein six ways from Sunday,” says Yale’s Candice Paulsen. “But they had never seen it.”
For a molecular biologist, seeing the structure of a molecule is revelatory. Suddenly you grasp how the thing is put together – and how you might heal it or cripple it or use its parts for other purposes. Such scrutiny allows researchers to know a molecule intimately, pinpoint its vulnerabilities, and promptly find drugs that target it by testing up to billions of compounds virtually using computer simulations.
Until recently, the best way to image molecules was with X-ray crystallography; scientists coax a molecule to form a crystal, blast it with X-rays, and then reconstruct its structure from the radiation patterns. When Erhu Cao, PhD, joined Julius’s lab in 2007, he tried to use this technique with the capsaicin receptor, to no avail. “He toiled away for three, almost four years, trying to get crystals,” Julius says. “Many proteins are just very difficult to crystalize,” explains Cao, now a biochemist at the University of Utah. He needed a new tool.
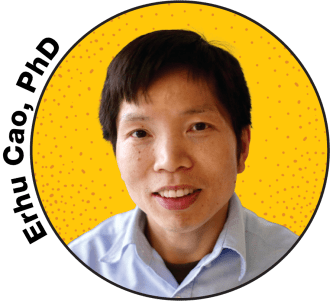
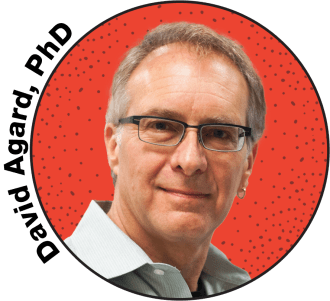
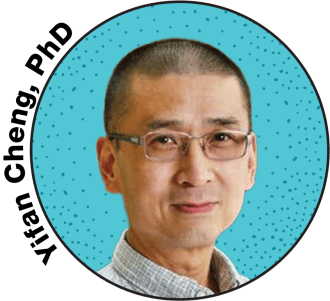
Meanwhile, two floors above Julius’s lab, UCSF biophysicists David Agard, PhD, and Yifan Cheng, PhD, were working to improve what was then a relatively obscure technology called cryo-electron microscopy, or cryo-EM. Invented in the 1970s, cryo-EM uses beams of electrons fired at frozen molecules to photograph their structures. For decades, however, the resolution was laughably poor. Most photographs came out looking like blobs, Cheng says. “That’s why, at the time, people doing cryo-EM were called ‘blobologists.’”
The cryo-EM pioneers believed they could do better. Digital cameras had made the process more efficient, but because the first-generation cameras worked with light, the electrons had to be converted to photons, which blurred the images. Hence the blobs. So Agard and his colleagues, including scientists at Lawrence Berkeley National Laboratory and the Massachusetts Institute of Technology, set about building a new kind of camera that detects electrons directly, resulting in sharper pictures. Agard enlisted Cheng to test and refine the prototypes, and Julius’s lab provided the perfect subject: the capsaicin receptor.
By the end of 2013, the team had published the first cryo-EM portraits of the famed receptor. The resolution was so good that you could make out the placement of each atom. “That sent a shock wave through the field,” Cheng says, because it showed that medically important molecules that resisted crystallization, like the capsaicin receptor, could be imaged with cryo-EM. “Almost every structural biology lab switched to doing cryo-EM overnight,” he says.
Today, more than 90% of molecular structures are solved using cryo-EM – including viruses. “After the first SARS outbreak, in 2003, it took years to work out the virus’s structure using X-ray crystallography,” Cheng points out. “With SARS-CoV-2, it took weeks.” Detailed cryo-EM images have allowed experts to track the evolution of coronavirus variants and quickly develop vaccines and therapies. “All this was done thanks to cryo-EM,” Cheng says. “If we did not push for this technology – if the field did not progress in this way – our understanding of the virus and our capability to fight it would lag way behind.”
Survival
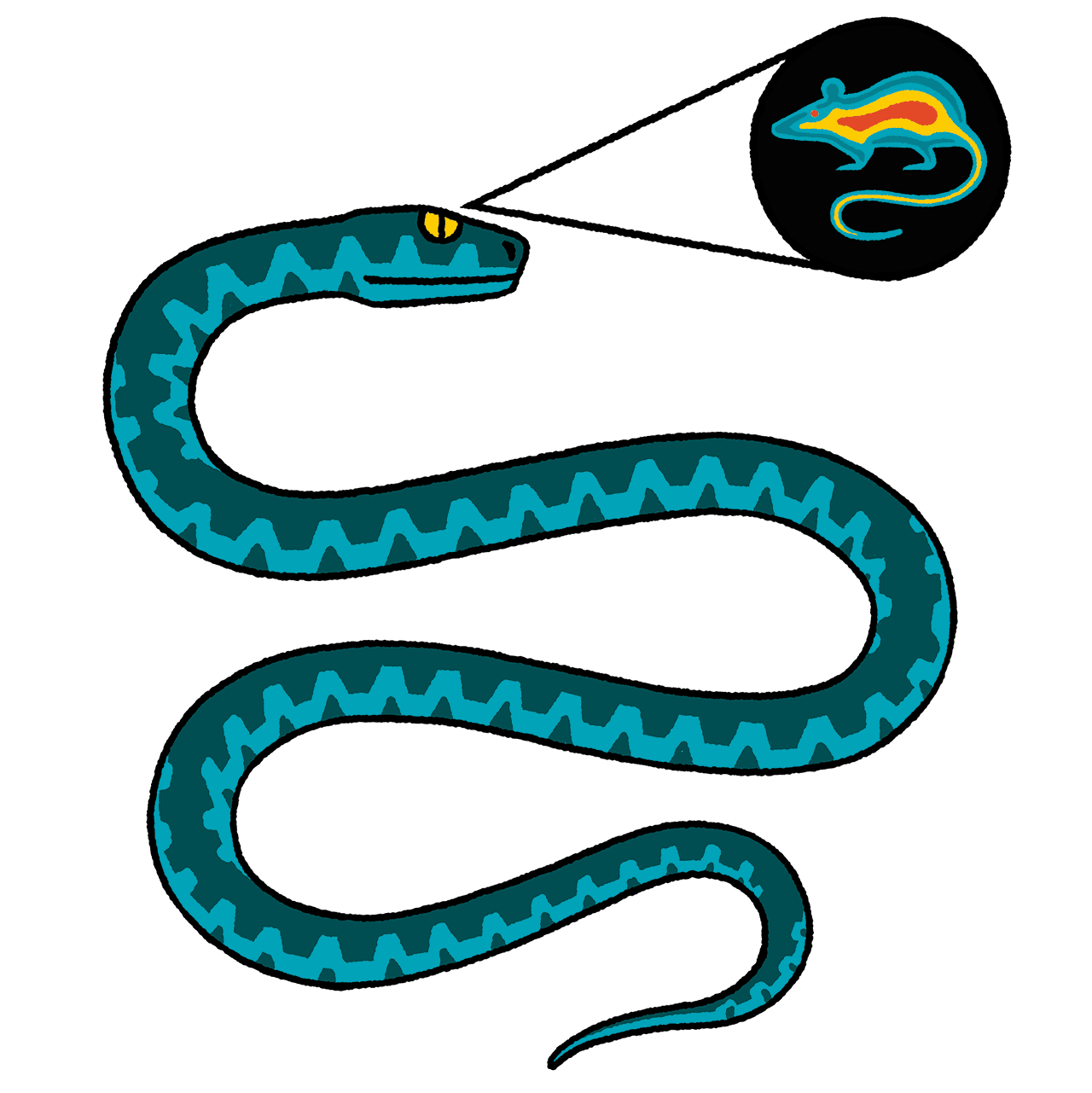
Super Sensors
When Elena Gracheva, PhD, was a fellow in Julius’s lab, she went to Texas to dissect some rattlesnakes. In a building with no windows (“so the snakes didn’t escape”), she cut from each viper’s brain a bundle of nerves projecting to organs in its face called pits. Pits allow rattlers and other snakes, including boas and pythons, to “see” the warm bodies of their prey via infrared radiation. Gracheva carried the snakes’ neurons in a cooler back to UCSF, where she isolated the molecule responsible: TRPA1.
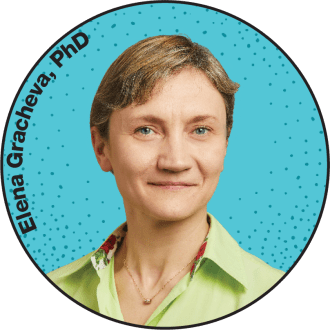
In humans and other mammals, TRPA1 plays a role primarily in sensations of pain and itch. (See “The Promise of Better Painkillers” and “Why We Scratch.”) But in snakes, the receptor evolved to detect radiant heat. “This is the beauty of evolution,” says Gracheva, now a physiologist and neuroscientist at Yale. “One molecule can take on many different functions.” She has since discovered other extraordinary adaptations, which, besides being just plain cool, could reveal ways of tweaking sensory molecules for therapeutic purposes.
Hibernating squirrels and hamsters, for example, have evolved TRPM8 receptors that are not sensitive to cold, as they are in humans, enabling these creatures to survive frozen winters quite comfortably. Similarly, a modification in the receptor TRPV1, which in humans responds to relatively modest heat, allows desert camels to withstand extremely hot climates. Another variant of TRPV1 endows vampire bats with an infrared sense like that of rattlesnakes. This sense is so acute that the blood-drinking bats can make out veins under the skin of their prey.
Beyond Sensation
One of the next frontiers of sensory-receptor science is understanding the impact of these molecules on human health beyond perceptions of pleasure or pain. For instance, piezo channels, which detect pressure, also regulate bodily functions like blood circulation and bone maintenance that have nothing to do with conscious feeling. Meanwhile, TRP channels, the family of receptors that sense temperatures, also contribute to heart rhythm, kidney filtration, insulin secretion, immune response, and the development of cancer, among many other processes.
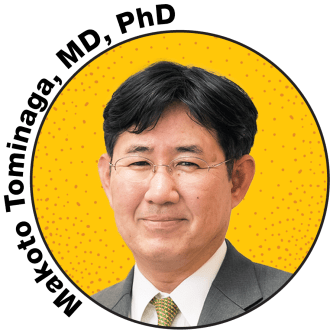
Physiologist Makoto Tominaga, MD, PhD, of Japan’s National Institute for Physiological Sciences – an alum of Julius’s lab who helped identify the capsaicin receptor, TRPV1 – is investigating the role of temperature receptors in brain repair. Recently, his team showed that when temperatures in the brain rise in response to an injury, support cells called glia sense the change via TRP channels and rush to fix the damage.
“There are sensory receptors regulating the gut, probably regulating development, procreation, spermatogenesis, you name it,” says UCSF’s Allan Basbaum. “The list goes on and on, and there’s a lot more to discover. We’re just at the tip of the iceberg, really.”
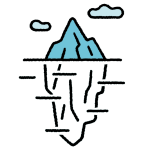