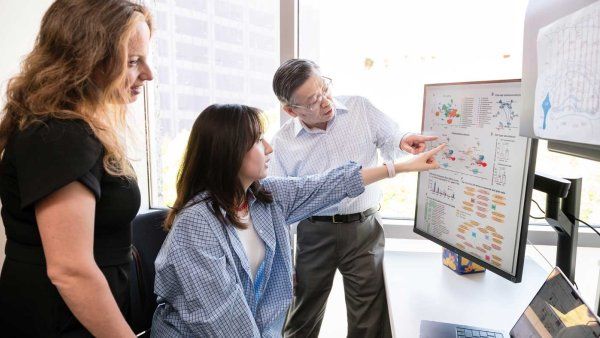
For as long as life has existed, so has the potential for disease. Four billion years ago, as meteorites pummeled the planet and volcanoes spewed toxic gases into the primordial atmosphere, a molecule emerged that could copy itself – a kind of proto-DNA. This was the dawn of evolution. It also marked the beginning of genetic disorders, since replication inevitably leads to copying errors.
Soon, the first organisms were born, and the process of cellular reproduction laid the foundation for aging. Later, the genesis of multicellularity – which gave rise to bodies, limbs, and organs – paved the way for cancer. And when immune systems evolved to fight off pathogens, they set the stage for asthma and autoimmune conditions.
Eventually, evolution forged the human genome. When our ancestors diverged from other apes, they acquired extraordinary capabilities, including language and tool-building. But their larger brains, upright postures, and lack of body hair also left them vulnerable to certain neurological, joint, and skin ailments. As human populations spread around the globe, they faced new environments and evolutionary pressures. A great diversity of genetic variation arose – and with it, a great diversity of new disease risks, including diabetes, migraines, and sickle cell disease.
By looking to humans’ evolutionary past, scientists at UC San Francisco are discovering not just how we get sick but why. They are finding that some of the same adaptations that make us uniquely human also make us uniquely susceptible to illness, particularly mental illness. They are learning how Neanderthals and other archaic human relatives passed along both advantages and disadvantages that many of us still harbor in our genomes today. And they are decoding the complex genetic legacy of humanity’s growth and migration over the past 100,000 years.
Our archaic human relatives passed along both advantages and disadvantages that many of us still harbor in our genomes today.
These roots carry profound implications for modern medicine. Already, they are revealing possible origins of psychiatric disease, heart disease, and other human maladies whose causes have long remained elusive. They are also advancing our understanding of disease risk, showing how each human’s distinctive ancestry can influence our health. This could all ultimately lead to new gene therapies and other personalized treatments inspired by ancient molecular innovations written in our DNA.
The Human Code
When scientists published the first human genome in the early 2000s, Katherine Pollard, PhD, was a graduate student in biostatistics. To the untrained eye, the genomic sequence might have looked unremarkable – just a jumble of As, Cs, Ts, and Gs strung together like tiles in an impossibly long Scrabble rack. But Pollard knew those four humble letters, which represent the chemical bases of DNA, spelled out the instructions for life. She had long been fascinated by humans’ origins. Now, with the blueprint for an entire person in hand, she was eager to compare it to that of our closest living relative, the chimpanzee. “I wanted to know what set us apart,” says Pollard, who today is a computational genomicist at UCSF and the UCSF-affiliated Gladstone Institutes.
Humans’ and chimps’ development diverged around 6 million years ago, but remarkably, their genomes remain nearly identical – only about 1% of our DNA is different. Many of these differences are random, having little to no effect on our biology. Others hold secrets to human nature, and Pollard was determined to find them.
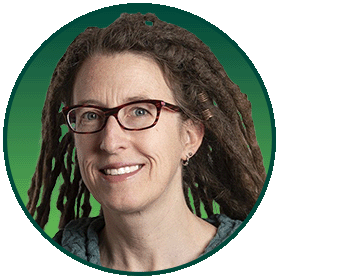
Professor of Epidemiology and Biostatistics
During her postdoctoral fellowship, in 2005, she wrote a computer program that scanned the human and chimp genomes, searching for the segments that diverged the most. She found more than 200, each less than a few hundred letters long. In chimps, these DNA strings are similar to those found in other vertebrates, including mice, fish, and chickens. This implies that for millions of years of vertebrate evolution, the strings had been frozen in time. Then in humans, they suddenly transformed. Pollard called these fast-evolving parts of our genome “human accelerated regions,” or HARs.
As the technology for sequencing and analyzing genomes improved, the list of HARs climbed to nearly 3,000. Pollard figured that because HARs had stayed the same in so many species before changing dramatically in humans, they must do something important – and that because they’re important, they must be parts of genes. But as Pollard and others were surprised to learn, HARs turned out to be something else.
Investigating the human genome is like exploring a vast library. Perusing the stacks, you might spot a thick, leatherbound volume – a gene. Inside is the recipe for a protein, a building block of living things. Browsing further, however, you might notice that such vital tomes are curiously scarce – a mere 2% of the library. Until recently, the remaining 98% – called noncoding DNA – was largely written off as junk, the evolutionary equivalent of credit card offers and campaign flyers that no one had bothered to toss.
This is where HARs reside. Their discovery helped convince the genetics community that such noncoding regions were worth a closer look. But if HARs weren’t parts of genes, what were they?
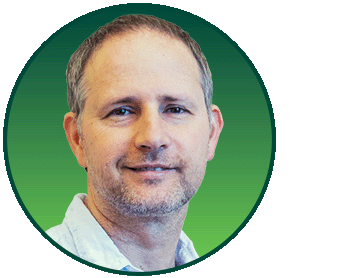
Professor of Bioengineering
Over the past decade, Pollard has been working with UCSF geneticist Nadav Ahituv, PhD. He directs the UCSF Institute for Human Genetics, and his lab had developed techniques to test hundreds of HARs in parallel. Their experiments using human and chimp cells have confirmed what Pollard and others had begun to suspect: Most HARs are regulatory sequences – manuals for how genes should operate.
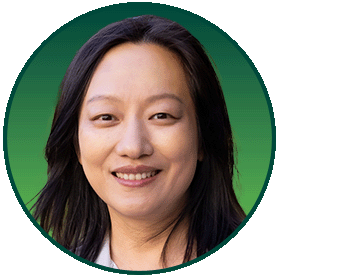
Professor of Neurology
Regulatory DNA tells a gene how many copies of a protein to make, in what cell types, and when. “It’s how your cells know what kind of cells to become,” says Yin Shen, PhD, an expert in gene regulation at the UCSF Weill Institute for Neurosciences and a collaborator of Pollard’s in the effort to decipher what HARs do and how they work. Many HARs, for instance, sit near genes involved in brain development, leading researchers to conclude that they might play a role in psychiatric disease.
Humans suffer from an array of neurological conditions like autism, schizophrenia, and Alzheimer’s, which chimps either don’t get or experience much less severely. People with these diseases are also more likely to carry HAR mutations. “Psychiatric research has been laser-focused on genes,” Pollard says. “There’s a growing realization that we need to be looking at regulatory elements, too.”
She and her colleagues have shown that HARs help orchestrate neural development, with about a third having unique functions in the human brain. Each HAR has between a few and a couple dozen DNA letters that changed during human evolution. Within a single HAR, some of the edited letters dial up protein production in human neurons, while others dial it down. These opposing effects suggest that many HAR edits evolved to offset others that had proven harmful. An edit that increased cognition, for instance, may also have raised the risk of psychiatric disease, leading to another edit that lowered the risk, like a game of tug-of-war.
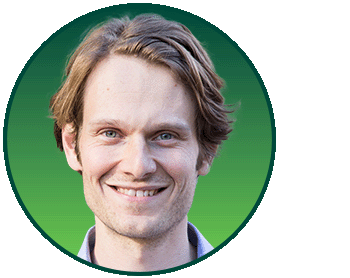
Associate Professor of Neurology
“Evolution involves tradeoffs,” says Alex Pollen, PhD, a neuroscientist at the UCSF Weill Institute for Neurosciences and another collaborator of Pollard’s. His lab grows brain organoids, tiny models of neural tissue, from human and great ape cells. He’s particularly interested in the genetic changes that drove the human brain’s dramatic enlargement.
“One of the special properties of the mammalian brain is that when it expands, not all of it expands equally,” Pollen says. He believes this uneven growth may have enabled cognitive advances but also created “vulnerable joints,” where some neurons had to work harder to sustain more or longer connections. The brain may then have evolved mechanisms to compensate for those weaknesses.
Pollen’s team recently found evidence for this theory in organoid models of Parkinson’s disease. “We think there are adaptations that are partially protective,” he says. “This may explain why we don’t all have Parkinson’s and could point us to new therapeutic targets.”
Our Neanderthal Inheritance
About 700,000 years ago in Africa, a branch of the human family tree split in two. One lineage stayed on the continent and evolved the anatomy of modern humans. The other migrated north, into Eurasia. Its descendants grew short and stocky, with big, wide noses, sloping foreheads, and prominent brows. These were the Neanderthals and Denisovans.
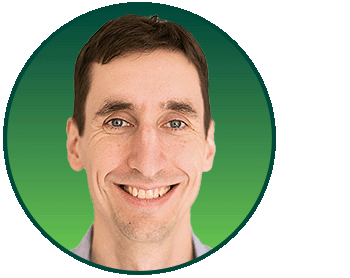
Professor of Epidemiology and Biostatistics
John “Tony” Capra, PhD, was a postdoctoral fellow in Pollard’s lab shortly after scientists figured out how to extract and analyze ancient DNA from human fossils. Not long after, journals began publishing the genome sequences of Neanderthals and Denisovans who lived tens of thousands of years ago. When waves of modern humans eventually left Africa, they interbred with these archaic cousins. Neanderthals and Denisovans have since vanished, but, as researchers discovered, traces of their DNA live on in us. Depending on your ancestry, up to 4% of your DNA could be Neanderthal and up to 6% could be Denisovan.
“That just blew my mind,” Capra says. He began to wonder: How do the fragments of archaic human DNA still lurking in our own genomes affect us?
At Vanderbilt University – where Capra first ran his own computational genomics lab before moving it to UCSF in 2020 – he and his colleagues embarked on a groundbreaking study. The results, drawn from a database of 28,000 Europeans, linked snippets of Neanderthal DNA, called variants, to a variety of health conditions. They found that inheriting combinations of Neanderthal variants increased an individual’s risk for depression and for getting skin lesions from being out in the sun. The team also identified specific Neanderthal variants associated with higher risk for tobacco use, malnutrition, bladder problems, and excessive blood clotting.
Other Neanderthal and Denisovan variants seem to be beneficial. Many people, for instance, carry archaic variants that affect immunity and metabolism, which may have helped their ancestors adapt to new pathogens and foods. Neanderthals also passed along variants for lighter skin and hair – a survival advantage at higher latitudes, where sunlight is weaker. And if you’re an early riser, it might be thanks in part to your Neanderthal or Denisovan inheritance, Capra recently discovered. Scientists believe that this trait may help the body adjust to seasonal daylight changes and reduce the risk of mood disorders.
Most archaic DNA, though, no longer exists in humans living today. “When we look at parts of our genome that control certain traits – cognitive traits, for example – there is way less Neanderthal contribution than we would expect,” says Capra, who is now a geneticist at UCSF’s Bakar Computational Health Sciences Institute. Many Neanderthal variants, he explains, must have disadvantaged the modern humans who inherited them. As a result, they were less likely to survive and reproduce, and so the variants gradually vanished from the human population.
Researchers debate what this meant for Neanderthals themselves. By the time modern humans encountered them, their numbers were dwindling. Smaller populations are more susceptible to genetic drift, in which rare variants can become dominant simply by chance, even if they’re harmful. Detrimental mutations could have easily piled up in the Neanderthal genome, Capra says. Neanderthals, he surmises, probably suffered from an array of ailments that could have led to their extinction. “I think they were a lot sicker than us,” he observes.
Beyond illuminating disease genetics, archaic genomes could be “prime hunting ground” for gene therapies, Ahituv says. In addition to HARs, he is studying the tens of thousands of variants that differ in the Neanderthal and Denisovan genomes and our own. Like HARs, many of the DNA strings that contain these variants are in noncoding regions that regulate gene activity. Testing these strings in human cells, Ahituv has begun to assemble a catalog of variants that produced different traits in modern and archaic humans by changing how genes behave.
As an example, he points to a DNA string that controls a gene called SATB2. The version of this sequence that existed in Neanderthals and Denisovans was stronger than ours – that is, it caused SATB2 to churn out more protein copies. The protein encoded by SATB2 helps shape the skull and brain; having more of it gave archaic humans a more prominent face.
SATB2, Ahituv notes, is also implicated in Glass syndrome, which, among myriad other symptoms, causes the opposite outcome: a face that’s unusually flat. If you wanted to create a gene therapy that reverses this effect – say, by designing a DNA sequence that boosts SATB2 expression – the archaic sequence could be a good one to try, Ahituv says. “It’s a crazy idea,” he admits, “but it shows how evolutionary history could be useful for clinical discovery.”
Map Source: Benton, et al. (2021), The influence of evolutionary history on human health and disease, Nature Reviews Genetics; Design: Frank Ramspott/Stephanie Koch
Map Source: Benton, et al. (2021), The influence of evolutionary history on human health and disease, Nature Reviews Genetics; Design: Frank Ramspott/Stephanie Koch
New Adaptations, New Risks
Humans have come to inhabit nearly every environment on Earth – from frozen tundras to tropical rainforests to arid deserts. As ancient peoples settled all around the world, their genomes continued to shape-shift, acquiring new DNA modifications that boosted their survival. But, as throughout evolutionary time, these adaptations often incurred tradeoffs.
In west-central Africa, where malaria is rampant, a gene variant molded red blood cells into the shape of a sickle and prevented infection by malaria parasites – giving rise to sickle cell disease. In the Himalayas, another variant enabled humans to function on less oxygen – but also elevated their blood pressure. In Scandinavia, a variant that increased tolerance to cold also triggered migraines. Sometimes, variants that were once assets became liabilities when a population’s descendants moved elsewhere or their circumstances changed. Genes that likely helped early Pacific Islanders store fat for times when food was scarce, for example, became risk factors for obesity and diabetes when calories became more abundant.
Other times, different genetic variants brought about the same diseases in different populations. This is particularly true of complex diseases like asthma or breast cancer, which involve hundreds or thousands of genes and regulatory regions. Most of what’s known about these diseases comes from genome-wide association studies (GWASs) – massive statistical analyses that compare DNA from many people to look for variants that are more common in those who have a particular condition than in those who don’t. The findings can then be used to calculate a person’s likelihood of developing that condition, known as their polygenic risk score.
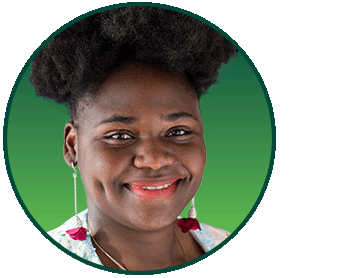
Assistant Professor of Epidemiology and Biostatistics
Polygenic risk scores are often unreliable for people of non-European ancestry because most DNA samples available for research have come from Europeans. But that is beginning to change. In 2022, Catherine Tcheandjieu, DVM, PhD, a UCSF and Gladstone genetic epidemiologist, published the largest and most diverse GWAS of coronary artery disease and the first to include a large sample of genomes from Black and Hispanic populations. The study found 95 new DNA regions associated with the disease, which greatly improved the accuracy of risk scores for people across the board – except Black people.
Tcheandjieu was puzzled. “You would think that if the biology of a disease is the same, you should be able to find the same mutations across populations,” she says. But many of the genetic variants previously associated with coronary artery disease didn’t show up in the Black population data. Maybe the problem was sample size: Did she just need more genomes to pick up a clear trend? Tcheandjieu didn’t think so. She had a hunch that something else was going on.
She decided to look closer at one of the suspect DNA regions, known as the heart attack gene. Although we all carry a version of this gene, the letters in it can vary, like alternate spellings of the same word. In non-African populations, many people with coronary artery disease share the same misspellings.
But when Tcheandjieu examined the heart attack gene in people of African ancestry, she observed a much greater variety of spellings in that population overall. This makes sense from an evolutionary perspective, she says. Because African populations are evolutionarily older than other populations, they have had more time to accrue more genetic variation. Such diversity, Tcheandjieu says, could explain why it’s been harder to find common risk variants in people with more African DNA.
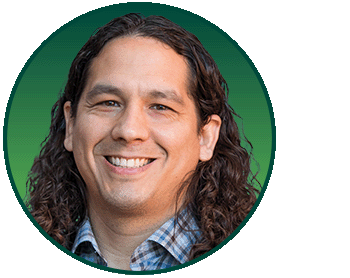
Professor of Bioengineering
Ryan Hernandez, PhD, a population geneticist at the UCSF School of Pharmacy, has found that Asian populations also have highly diverse genomes, but for a different reason. “In Asia, there have been massive population booms, where billions and billions of people have emerged over a very small amount of evolutionary time,” he explains. Because each birth has supplied dozens of new mutations, rare variants have accumulated quickly.
Hernandez, who runs a lab with UCSF evolutionary geneticist Dara Torgerson, PhD, is working to understand the cumulative effects of rare variants and to develop new analytical tools to study genomes that don’t fit the European mold. This is especially challenging in the Americas, he says, where many people have admixed ancestries. “I myself am admixed,” he says. “My father is Mexican American, and my mother is white. My genome includes bits of DNA that came from Europe, bits from Africa, bits from American Indigenous populations. So I want to know: How the heck do I study me?”
My father is Mexican American, and my mother is white. My genome includes bits of DNA that came from Europe, bits from Africa, bits from American Indigenous populations. So I want to know: How the heck do I study me?”
The human story is still unfolding. Every baby born is a chance to tweak the genetic code. These revisions might be strengths, making the people who inherit them a little fitter or more resilient. Or they might introduce new disabilities or ways of suffering. Or possibly both at the same time. And as our environments and societies change, what was once beneficial might become harmful, or vice versa.
What remains to be seen is whether we can harness these evolutionary forces to build a healthier future.